Molecular magnetism
Single-Molecule Magnets (SMMs) have been investigated extensively as a result of their potential applications in high density data storage. Since the discovery of the first SMM in 1993, the hysteresis temperature (i.e. the temperature at which molecular magnets display magnetic remenance) only increased from 4 K to 14 K. In 2017 in collaboration with the group of Dr Nicholas Chilton we reported the dysprosocenium complex 1 (Figure 1), which displays magnetic hysteresis at temperatures as high as 60 K (Nature, 2017, 548, 439). This lanthanide SMM is only 17 K from liquid nitrogen temperatures, at which point real-world applications of SMMs could become a possibility, e.g. data storage devices.
Single-Molecule Magnets (SMMs) have been investigated extensively as a result of their potential applications in high density data storage. Since the discovery of the first SMM in 1993, the hysteresis temperature (i.e. the temperature at which molecular magnets display magnetic remenance) only increased from 4 K to 14 K. In 2017 in collaboration with the group of Dr Nicholas Chilton we reported the dysprosocenium complex 1 (Figure 1), which displays magnetic hysteresis at temperatures as high as 60 K (Nature, 2017, 548, 439). This lanthanide SMM is only 17 K from liquid nitrogen temperatures, at which point real-world applications of SMMs could become a possibility, e.g. data storage devices.
Figure 1. Structure of 1.
This result is in line with previous predictions that highly axial ligand fields can maximise the magnetic properties of Dy(III) to give SMMs with the highest energy barriers to the reversal of magnetization, including our work on the first near-linear f-element complex 2 (Figure 2) (Chem. Commun., 2015, 51, 101). We are carrying on our investigations of highly axial lanthanide SMMs in close collaboration with the group of Dr Nicholas Chilton and the Molecular Magnetism Group. We are currently targeting new lanthanide SMMs that could operate above liquid nitrogen temperatures (J. Am. Chem. Soc. 2017, 139, 18714).
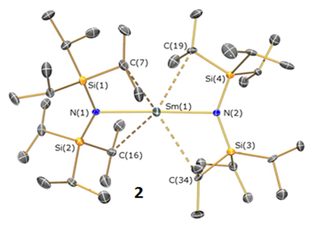
Figure 2. Structure of 2: a blueprint for a linear Dy(III) high temperature SMM.
Low oxidation state f-element chemistry
Over the last three decades there has been a renaissance of f-element chemistry, which has led to the discovery of new oxidation states for lanthanides and actinides. Some of this work was inspired by Lappert’s seminal report of the first structurally authenticated Th(III) compound in 1986 surrounded by three sterically demanding cyclopentadienyl ligands. In 2017 we reported a new Th(III) tris-Cp complex 3 (Figure 3) and its U(III) analogue and ran sophisticated pulsed EPR measurements in collaboration with Prof. Eric McInnes and Dr Floriana Tuna (Nature Chem., 2017, 9, 578). The interpretation of these data sheds light on the ligand-metal interactions for Th and U, providing new insights on the long-debated question of the importance of covalency in the actinide series. As the reactivity of Th(III) compounds are relatively underexplored, we are currently investigating the reactivity of 3 and related compounds with small molecules, such as CO2, SO2 and P4 (e.g. Chem. Eur. J., 2016, 22, 17976).
Over the last three decades there has been a renaissance of f-element chemistry, which has led to the discovery of new oxidation states for lanthanides and actinides. Some of this work was inspired by Lappert’s seminal report of the first structurally authenticated Th(III) compound in 1986 surrounded by three sterically demanding cyclopentadienyl ligands. In 2017 we reported a new Th(III) tris-Cp complex 3 (Figure 3) and its U(III) analogue and ran sophisticated pulsed EPR measurements in collaboration with Prof. Eric McInnes and Dr Floriana Tuna (Nature Chem., 2017, 9, 578). The interpretation of these data sheds light on the ligand-metal interactions for Th and U, providing new insights on the long-debated question of the importance of covalency in the actinide series. As the reactivity of Th(III) compounds are relatively underexplored, we are currently investigating the reactivity of 3 and related compounds with small molecules, such as CO2, SO2 and P4 (e.g. Chem. Eur. J., 2016, 22, 17976).
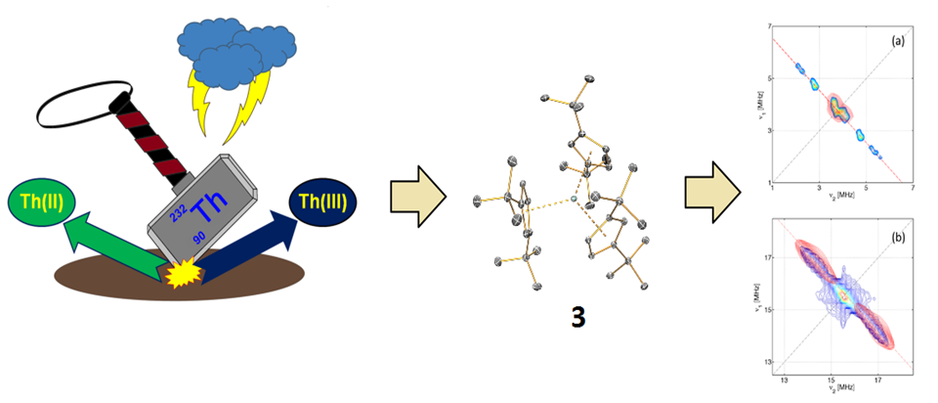
Figure 3. Structure of 3 with pulsed EPR spectra.
Although the +2 oxidation state for lanthanide solution chemistry was previously limited to samarium, europium and ytterbium, the last 20 years has seen a renaissance in this area. We have access to a bespoke reactor, one of only a handful worldwide, that allows us to routinely prepare +2 precursors for neodymium, dysprosium and thulium. In 2016 we reported the first thulium amido complex in the +2 oxidation state, 4 (Figure 4, Inorg. Chem., 2016, 55, 10057). We are currently investigating the synthesis and physicochemical properties of a wide range of low oxidation state lanthanide complexes
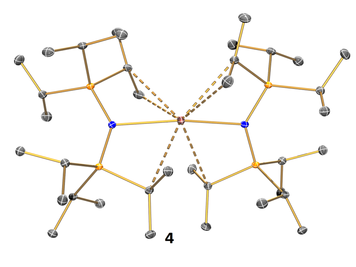
Figure 4. Structure of 4.